However, increased R&D efforts are needed to facilitate an efficient and safe scaling up of CCS to the level needed to mitigate climate change. In his keynote speech at TCCS-12, SINTEF Chief Scientist Svend Tollak Munkejord discussed why both experiments and models are a necessary part of this effort.
Painting a clear picture of CCS’ role in mitigating climate change
At the recent 12th Trondheim CCS Conference (TCCS-12), I had the honour of giving a keynote lecture on a topic I’ve worked a lot on – the safe design and operation of CO2-transport systems. Previous keynote speakers, in particular Kikki Flesche Kleiven from the Bjerknes Centre for Climate Research had painted a clear and frightening picture of the urgency of addressing the climate crisis. Here, as almost everywhere, there is not one easy solution – ample details have been presented by the International Panel on Climate Change (IPCC), the International Energy Agency (IEA) and others. In order to mitigate climate change, we need all the tools in the toolbox. CCS, that is, capturing CO2 that would otherwise be emitted, and storing it safely under tight rock formations, is one of those tools.
Painting a clear picture is the first step. The next step is to start building CCS systems. When the engineers start laying out the details, they need to assign numbers to things. If the numbers are unknown or uncertain, the engineers need to put larger safety margins into the designs. While current engineering tools can do a lot, they cannot do everything we would like them to do in the CCS domain. This does not mean that CCS cannot be done, only that the first systems are a bit clumsier than the future ones will be. This is the main reason why we perform CCS research in NCCS and many other projects.
To illustrate this point, consider electric cars. Good and useful electric cars have existed for more than 110 years, such as the very nice 1911 Detroit Electric, with a range of 130 km and a top speed of 32 km/h. This is where CCS is today. It is good and useful, but more research and development are needed.
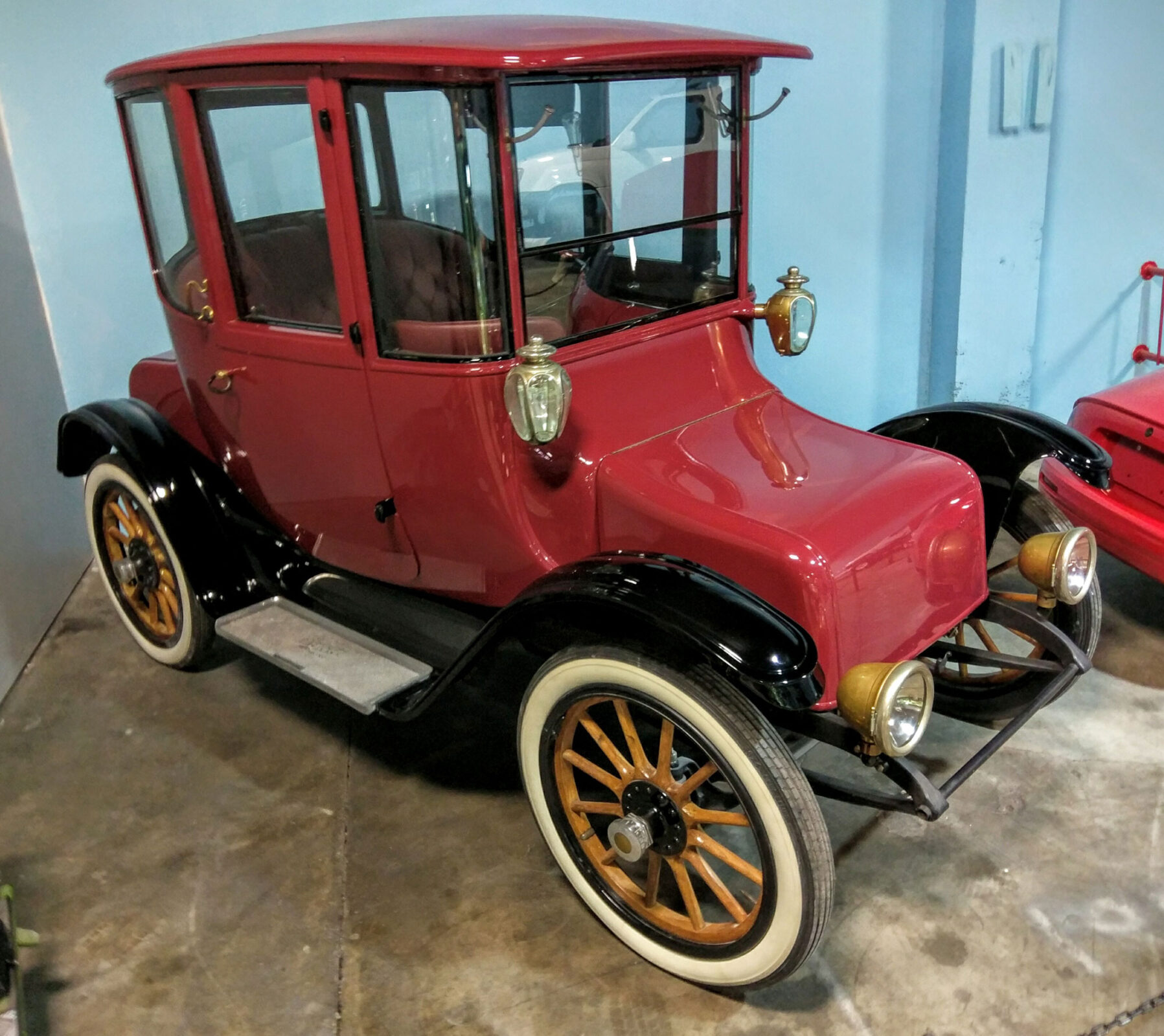
As researchers, we can provide the data, models and insights needed to deploy safe and efficient CCS systems capable of handling several gigatonnes of CO₂ per year by the mid-century. However, while it is true that firm knowledge can contribute to reducing costs, the fact of the matter is that no matter how cheap CCS may be, it will always be more expensive than to keep polluting – unless we also implement the necessary regulations. It is the responsibility of our leaders and governments to put such regulations in place.
Modelling and experiments go together: you can’t have one without the other
Because TCCS is a scientific conference, in my talk I took the liberty of being a bit technical. One key point is the relation between experiments and models: they are both needed. This has been understood since Galileo Galilei directed his telescope towards planets and stars, and confirmed the heliocentric model – but that does not mean that it is always easy. In fact, it is challenging to ensure that the experiments and models describe exactly the same thing. In this context, a model is a simplified view of a physical phenomenon or system, with accompanying equations to describe this view.
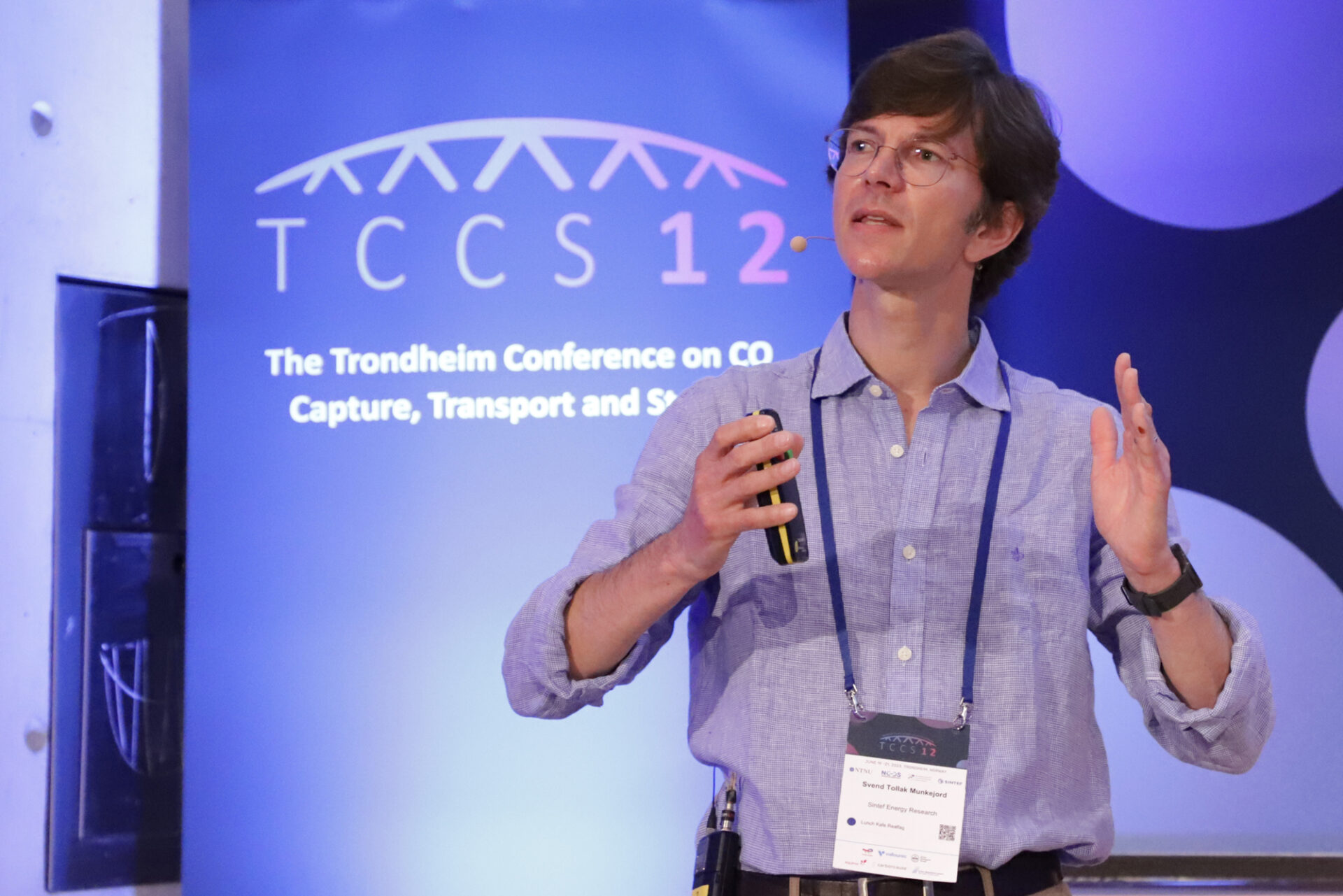
Consider now CO₂ flowing in a pipeline between the capture plant and the storage site. Depending on where the CO₂ is captured from, the CO₂ stream could include one or more impurities, such as nitrogen, argon, hydrogen or others. We can purify the CO₂ to a high degree, but this is expensive. So, we expect it is possible to find an optimal degree of purification, but the price to pay is to make sure the impurities do not cause trouble downstream. It turns out this is complicated because of the large number of impurities and their possible interactions. One main effect of impurities is the potential for corrosion. Another is that impurities influence the thermophysical properties of the CO2 stream, such as density, speed of sound and viscosity.
In a slightly simplified example, consider a CO₂ stream consisting of 1.8 mol % nitrogen in the CO₂. At a pressure of 120 bar and a temperature of 20°C, if the flow speed is the same, the nitrogen content means that the density will decrease by 3 % and the viscosity will decrease by 6.4 % with respect to the values for pure CO₂. We know that viscosity is a measure of the resistance with which a liquid flows, so this would mean that we can spend 6.4 % less pumping power, right?
Not quite, it turns out. I will not bother you with the details here, but we need to consider the flow equations for steady, turbulent flow. For my example, the equations show that the pressure drop will go down by 3.1 %, out of which only 0.2 % is due to the viscosity change; the rest is due to the density change. This is illustrated in the chart below.
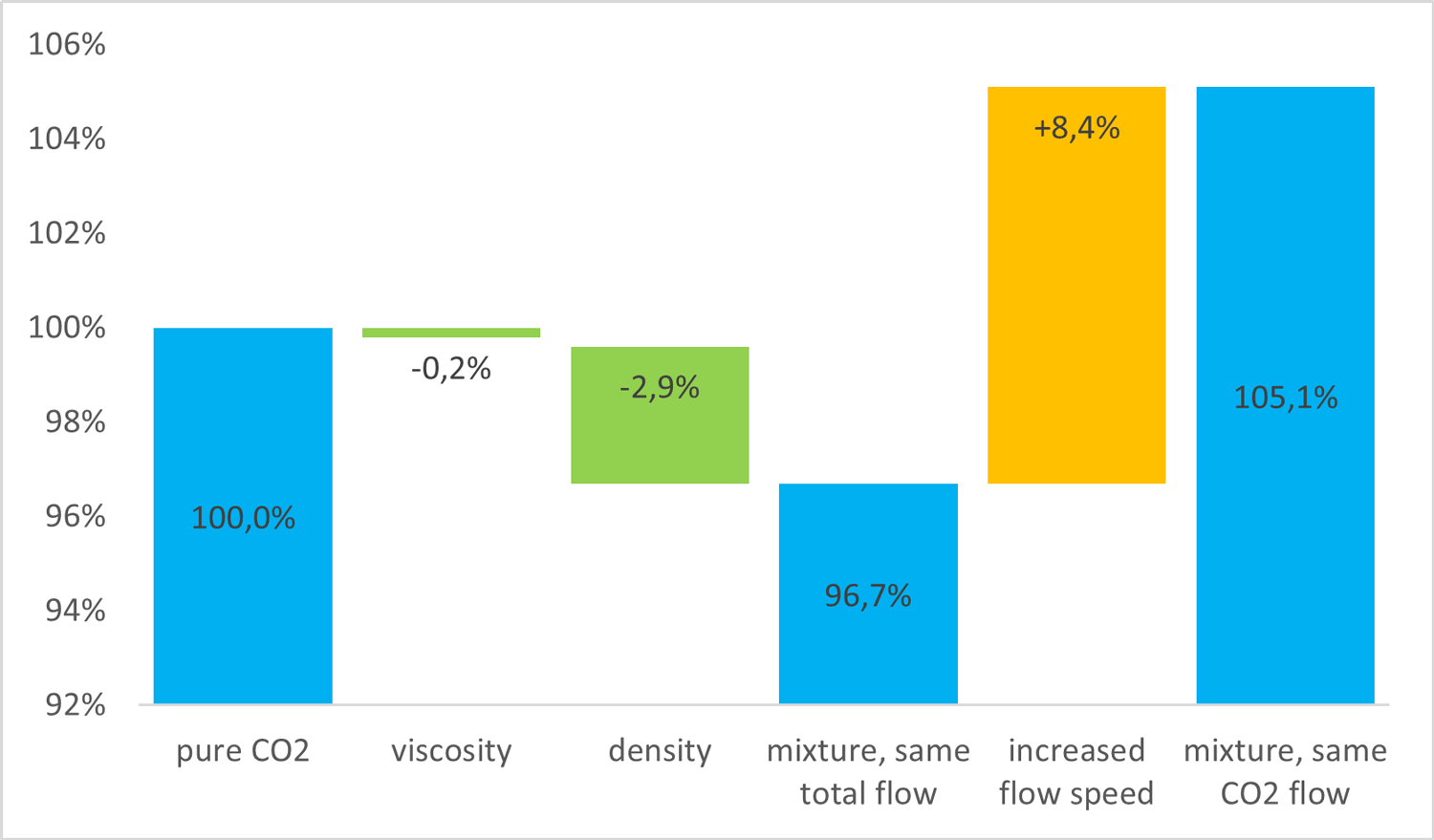
In addition, because we are interested in transporting and storing CO₂ and not impurities, we need to transport a larger total amount of fluid if we do not remove impurities. This gives a 5.3 % higher pressure drop.
Does this mean that viscosity is an unimportant quantity? Not at all, this was just an illustration that the ‘gut feeling’ is often insufficient; we have to do the relevant calculations – and modelling and experiments go together. You can’t have one without the other.
Designing our own setups for understanding CO₂ flow dynamics
When we want to perform experiments to understand the flow dynamics of CO₂, where the CO₂ state can change from liquid to gas to solid (dry ice), we cannot buy standard equipment; we have to design and build the setups ourselves. How the CO₂ state varies as a function of pressure and temperature is illustrated in the phase diagram below.
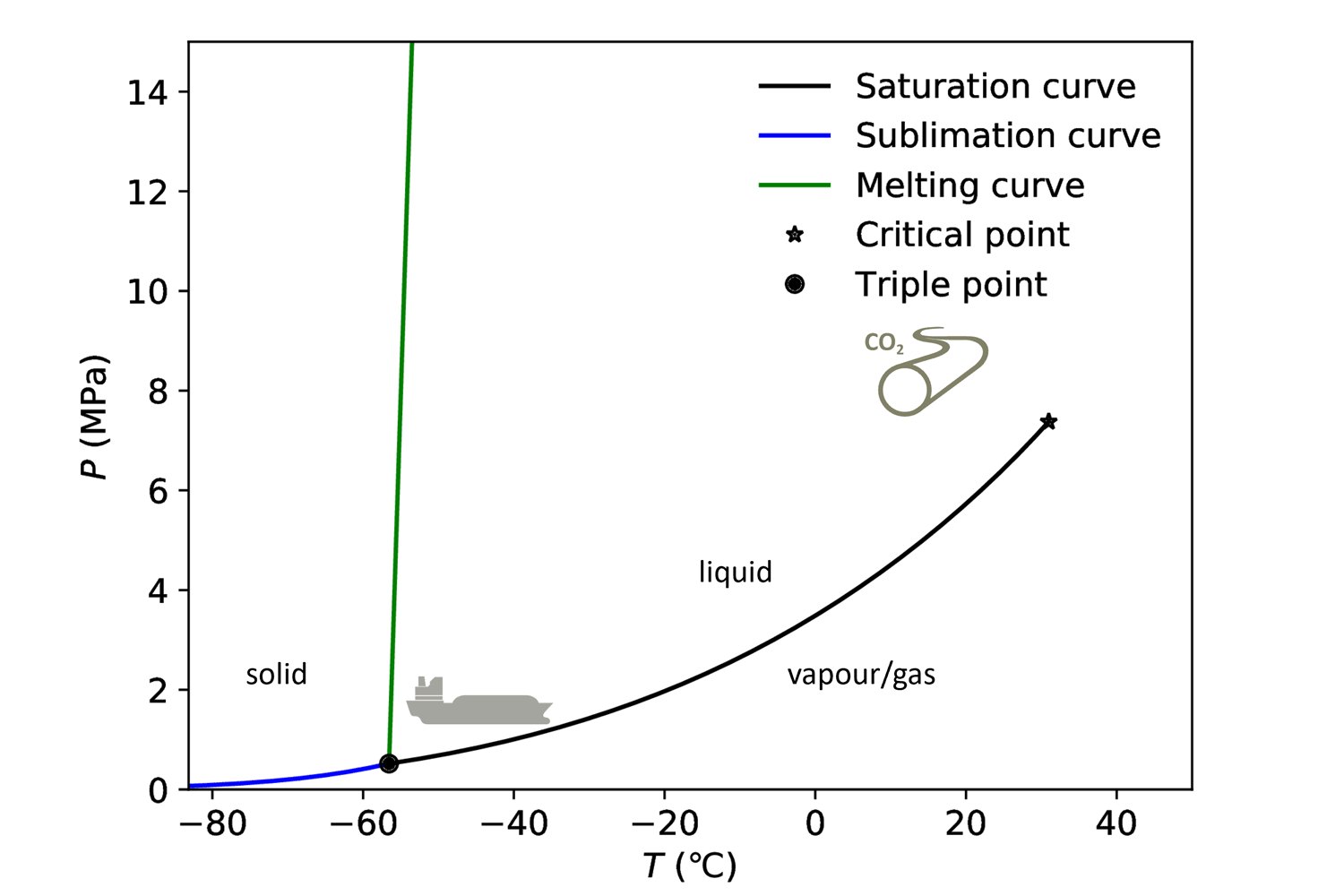
In our CO₂ transport laboratory, we have made several setups to study the coupled fluid- and thermodynamics of CO₂:
- ECCSEL FASafe – Flow assurance for safety systems. This setup has been designed to study the formation of solid CO₂ (dry ice) during load transfer and venting. More detailed understanding of dry ice behaviour will be useful, e.g., for optimized design and operation of CO₂ transport by ship. The setup is just becoming operational and we are looking forward to putting it to use.
- ECCSEL DeFACTO – Demonstration of flow assurance for CO₂ transport. This setup was built in the DeFACTO project with Equinor and TotalEnergies as partners. We use it to study vertical flow of CO₂ in a 90 m deep U-tube, and phase transfer (condensation or evaporation) can be included.
- ECCSEL Depress – Depressurization facility. In this facility we study depressurization flow behaviour of CO₂ in a pipe or in a vessel. Data from this facility has been published on Zenodo, and has led to a suggestion for an improved model for running ductile fracture, a key design issue for high-pressure CO₂ pipelines.
Read more: Why is understanding choked flow crucial for CO₂ capture, transport and storage?
With these and other efforts, we aim at providing engineers and scientists with data and models that are not otherwise available, in order to facilitate the efficient and safe scaling up CCS to the gigatonne level needed to mitigate climate change.
This work was performed in the Norwegian CCS Research Centre (NCCS), supported by industry and the Research Council of Norway.
Comments
No comments yet. Be the first to comment!