This spring, SINTEF and IFP Energies nouvelles has assessed the current knowledge on the potential for hydrogen in Europe.
The key question of the assessment was if strategic deployment of hydrogen in Europe can increase the probability of reaching its climate targets and at reduced costs compared to alternative paths with low deployment of hydrogen.
In the work, hydrogen from natural gas where the produced CO2 is captured and stored permanently has been given primary attention, but hydrogen from renewable sources such as biomass, wind and solar has also been assessed. Below you will find a summary of the main conclusions. If you are curious on the details behind we have also added those further down. Wish you a good read.
1 About the Hydrogen for Europe pre-study
The pre-study, commissioned by IOGP and IOGP’s member companies, has been carried out in the context of the ongoing process within the EU on how to fulfil the commitment made to the Paris agreement. Reaching climate neutrality, or near neutrality, by 2050 requires a significant increase in annual reductions of greenhouse gas (GHG) emissions compared to historic reductions from 1990 to 2016.
Hydrogen is an energy carrier which, as for electricity, has no greenhouse gas emissions related to it when being used. Further, hydrogen can be used in the industry, transport sector, power sector and to heat the building stock. Therefore, hydrogen could potentially be part of the solution to achieve the necessary decarbonization of Europe’s energy system. The Hydrogen for Europe pre-study was launched to study current knowledge on the potential for hydrogen in Europe. Particularly, the study investigated available knowledge on the potential role of hydrogen produced from natural gas where the CO2 produced is captured and stored and possible interplay with hydrogen produced from renewable sources. The study has assessed currently available knowledge with regards to what can be learned about the full potential of hydrogen in Europe and how future work could be carried out to gain more comprehensive knowledge about the potential.
Oil, salmon and …. liquid hydrogen – Norway’s next big export article?
In our work, three main sources have been used:
- “A Clean Planet for all” – In response to the Paris agreement and to confirm Europe’s commitment for efforts and actions to limit climate change, the European Commission adopted a long-term strategic vision for a prosperous, modern competitive and climate neutral economy by 2050 – “A Clean Plant for all” . The consultation document assesses eight different pathways, two of which aim to achieve climate neutrality by 2050. The pathways explore the opportunities and efforts needed to reach EU’s climate targets. They show the radical changes needed in the European energy system, which include the utilisation of hydrogen and synthetic methane as energy carriers.
- “Hydrogen Roadmap Europe” – A study on the potential of the European hydrogen market carried out by the Fuel Cells and Hydrogen Joint Undertaking and McKinsey. The study investigated two scenarios, Business as Usual and an ambitious scenario reaching the 2.0 °C scenario, and estimated the investment and technology needed to deploy hydrogen technologies.
- H21 North of England Report – The North of England project is a detailed analysis of hydrogen production and distribution in northern England for heating purposes. This study was conducted by Northern Gas Networks and Equinor to study the decarbonization of the heating sector. All fossil fuel-based production pathways include CCS. Hydrogen production technologies analysed include natural gas reforming in both a steam methane reformer (SMR) and an autothermal reformer (ATR), coal gasification, offshore wind-powered electrolysers, as well as storage of hydrogen in the form of ammonia. Furthermore, they investigated the required hydrogen transport network and the seasonal storage of hydrogen. The results of the different production routes can be compared due to similar assumptions in the report.
The assessment of the potential benefits of hydrogen in the energy transition should address three main questions:
- What is the potential for reducing GHG emissions in Europe using hydrogen?
- What is the cost of transitioning the European energy system, from today to 2050, and beyond? Of special interest is the relative cost of transition paths with different degree of hydrogen utilization and other paths with no use of hydrogen.
- What is the viability of energy transition paths? Relevant parameters for assessing the viability are technology readiness and possible deployment rates, policy and regulatory risks and barriers, technology safety and social acceptance.
In the following, we will give a summary of the main assessment results of the Hydrogen for Europe pre-study, in accordance with the questions above.
1.1 The potential for reduced GHG emissions in Europe due to the application of hydrogen
Hydrogen can be produced from electricity, natural gas, and biomass where biomass includes biogas. Biomass gas is already present in the European gas network. Biomass can also be used to produce hydrogen, through gasification and reforming processes, as illustrated in Figure 1. Electrolysers are used to produce hydrogen from electricity and water. Natural gas can be used to produce hydrogen through reforming processes, either steam methane reforming (SMR) or autothermal reforming (ATR). The CO2 produced can be captured and stored to significantly reduce the emissions from the production.
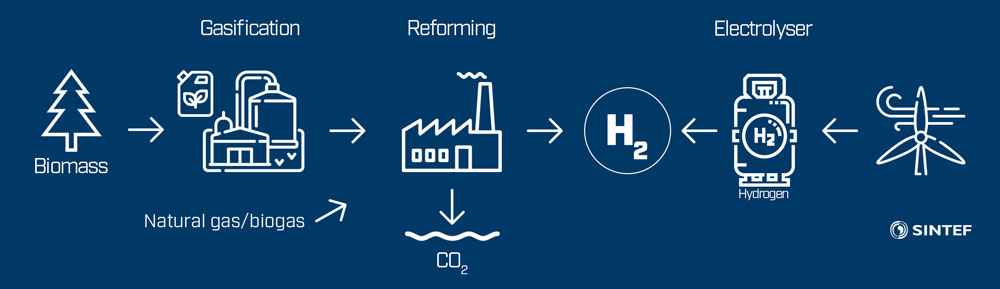
Figure 2 compares emissions from hydrogen production from grid electricity and from natural gas with CCS. The average European grid electricity has been chosen as the source for hydrogen production through electrolysis as a simplified approach to include the emissions from the power generation. In 2016, 43 % of the energy used to produce electricity were fossil without CCS[1]. Production of renewable energy dedicated to hydrogen production could potentially affect the decarbonization rate of the European power sector until the fossil fuels used to produce electricity have been replaced by renewable sources or the CO2 produced in the electricity generation is captured and stored. A future study should develop an increased knowledge about the effect of dedicating renewable energy to hydrogen production and how this affects the decarbonization of the power sector that differs across Europe and is predicted to have an increased demand.
As seen in Figure 2, emissions related to hydrogen production have been compared for three points in time. The result for 2016 is based upon an average European CO2 emission intensity of 296 kg CO2 per MWh[2] with a corresponding emissions rate of 393 g CO2 per kWh (based on the higher heating value) of produced hydrogen[3]. This is 7.7 times higher than for hydrogen produced from natural gas with CCS, where up- and mid- stream CO2 emissions have been included.
Outlooks from “A Clean Planet for all”[4] and IRENA’s “Renewable Energy Prospects for the European Union”[5], give a corresponding ratio in the range of 5.3 to 5.6 for 2030, compared to 7.7 for 2016. Hence it is reasonable to assume that well beyond 2030 the hydrogen with the lowest carbon intensity would be produced from natural gas with CCS.
The estimate for 2050 is made from the most ambitious scenario presented in “A Clean Planet for all” which reaches climate neutrality in 2050. It shows that by this time, the carbon footprint of electricity production is small enough to produce hydrogen with least emissions. The precise timing for this transition is uncertain and will depend on the pace of deep decarbonization of the grid post 2030. This pace could be affected by the future deployment rate of hydrogen and the sources it is produced from as e.g. high shares of hydrogen from renewably produced electricity increases the total electricity demand. Further details about the estimates presented in the figure below.
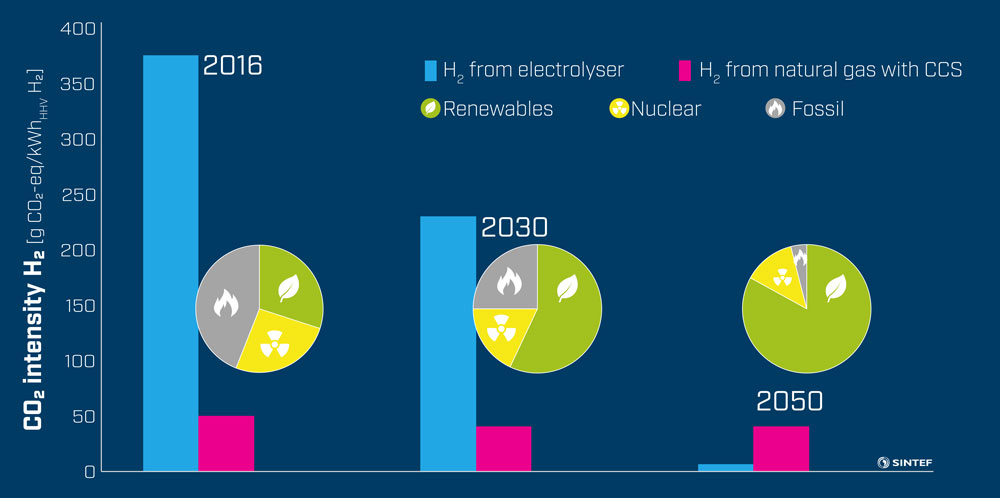
Figure 3 presents upper limits for reduction of European CO2 emissions by replacement of fossil fuels with hydrogen from natural gas with CCS in the power, residential and commercial, transport and industry sectors by 2050. The estimates are based on IEA statistical energy information, the European Commission’s Baseline forecast for 2050 of “A Clean Planet for all”, and outlooks from Hydrogen Roadmap for Europe. For each sector, the potential for hydrogen to replace the use of fossil fuels has been evaluated. For example, in the transport sector, only heavy-duty road transport and rail has been considered, in heating of building stock it has been assumed that hydrogen can be used to replace all use of fossil fuels. A forecast of the degree of deployment within each sector has not been included in the estimate, with the exception of the power sector, where a modest deployment has been assumed due to the expected future increase of electricity produced from renewable energy sources. This has been done to allow readers to perform individual assessments of deployment rates for each of the sectors.
Further details about the development of the potential for emissions reductions per sector can be found in Section 2.3.
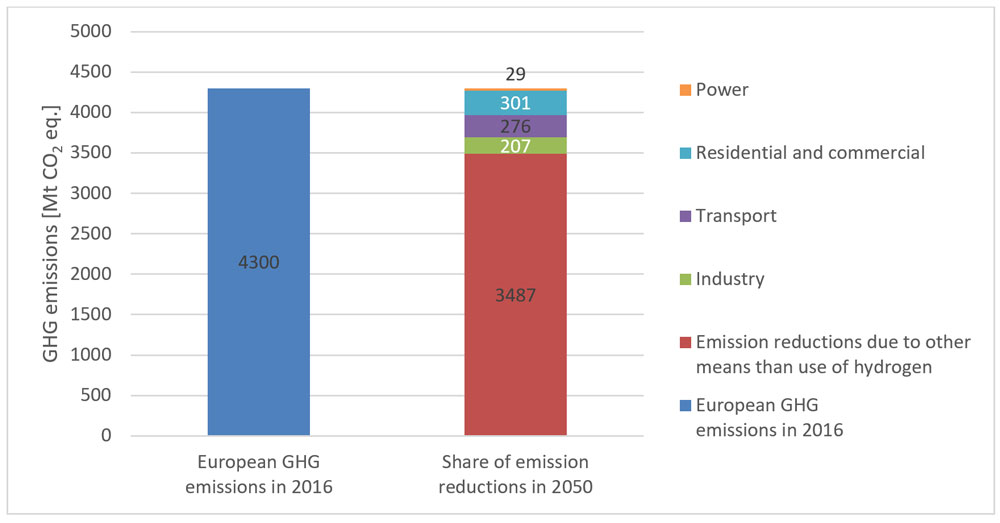
The assessment showed that the hydrogen potential is within the limits of the volume of hydrogen that can be produced from the natural gas currently consumed in Europe. The corresponding total annual GHG removal by 2050 was estimated to 813 Mt CO2 eq., or 19% of current GHG emissions in Europe.
A full overview of potential GHG emission reductions for different combinations of hydrogen sources should be derived in a future study.
1.2 The cost of the energy system transition, from today and until 2050 and beyond
Currently, “A Clean planet for all” is the most comprehensive study on the European energy system transformation. The study presents comparisons of overall costs of the selected scenarios. However, production of hydrogen from biomass and natural gas with CCS is not included. Hence, the relative costs of the transition paths that include hydrogen from natural gas with CCS have not been estimated.
The two scenarios of the Hydrogen Roadmap Europe include the use of hydrogen. However, the study does not contain any information on the relative cost compared to other viable pathways to decarbonization.
The H21 North of England project has focused on a specific geographical area and is therefore not readily applicable to the rest of Europe due to e.g. differences in sectorial energy demands, natural gas storage and distribution system and available storage sites for CO2. The data available in the report largely covers the need for technical information on hydrogen production and transport, including transport and storage of CO2, which is useful for a future European-wide study.
One observation made in the current pre-study is that hydrogen production from natural gas using CCS could be integrated into industrial clusters. This could
- Increase the volume of CO2 transported from the cluster to the storage location. If the cluster contains industries that can only be decarbonized with CCS, the additional volumes of CO2 could provide economies of scale benefits and reduce the CCS unit costs.
- Provide two ways to decarbonize the industrial cluster – hydrogen and direct implementation of CO2 capture at the industrial plant.
- Decarbonization of building stock heating, transport sector, power sector and other industrial plants through distribution of hydrogen in the network infrastructure.
A future study should assess whether such an integration of hydrogen production into industrial clusters has the potential to reduce the cost of decarbonization. Such a study should also investigate possible technology lock-out effects for hydrogen and CCS caused by limited technology investments in an early phase and the possible impact on overall costs of decarbonization.
In conclusion, no current studies have examined the comparable costs of viable paths to decarbonization such that it is possible to assess the potential for hydrogen in Europe. However, our overview shows that most of the data needed to conduct such a study are available. Additional information is mainly needed on the cost and efficiency of hydrogen appliances. A future study should also investigate the sensitivity of the estimated costs to the expected consumption costs for natural gas, biomass and electricity.
1.3 The viability of the energy transition paths
A “Clean Planet for all” included substantial information on the necessary deployment of technologies for the selected scenarios. The results are however to a limited extent investigated in terms of feasibility of these technologies e.g. technology maturity and realistic deployment rates.
The “Hydrogen Roadmap Europe” included an estimate of the investment and the technology needed to reach the development assumed in the scenarios. In both scenarios, the projections are based on a given set of market developments for the transport, industry, building stock heating and industry sectors. However, an in-depth discussion on the realism of the scenarios is not presented.
H21 North of England study assessed the feasibility of providing the electricity capacity required for hydrogen production. Within the H21 North of England project, it was concluded that the necessary installation of 18.4 GW electrolysers would not be feasible in the desired timeframe, even if large companies like ThyssenKrupp deploy their concept to a full extent. On the contrary, natural gas-based hydrogen production could be installed at this level within a limited time frame, due to a mature industry and the large-scale production of natural-gas based hydrogen in the chemical industry. The conversion of the natural gas grid and the domestic and industrial heating sector is, according to the report, ambitious, but achievable.
Based upon the current findings, there is a need to better understand the feasibility of deployment rates of hydrogen appliances as well as technologies related to hydrogen production and energy storage concepts such as batteries and large-scale hydrogen storage.
In addition to the technical viability of hydrogen deployment cases, barriers to the use of hydrogen from natural gas has been investigated in the current pre-study. The results of the HyLaw project were the main source of the investigation. It was found that many of the barriers to hydrogen deployment result from regulatory gaps caused by a lack of harmonization of rules and approaches at European level. However, EU legislation increasingly refers directly to hydrogen and has a major impact on the deployment of hydrogen technology, especially on the use of hydrogen as a fuel.
In all EU countries barriers are present but their severity varies. In any case, actions are necessary to unlock the full potential of hydrogen technologies in all countries and at EU level. Relevant authorities should review technical and gas composition rules to establish legal pathways to support Power-to-Gas operations and increase hydrogen use in transmission and distribution gas networks.
It is essential to carry out a coordinated EU wide review of the safety and technical integrity limitations for the injection of hydrogen into the gas grid. A more comprehensive overview of barriers is presented in Section 2.6.
ELEGANCY Dutch Case Study: TNO initiates industrial participation with H-Vision project
1.4 References
- A Clean Planet for all by the European Commission (2018).
- Hydrogen Roadmap Europe by FCH-JU (2019)
- H21 North of England (2018)
- Energy statistical datasheets for the EU countries from Eurostat (2018)
- Renewable Energy Prospects for the European Union, IRENA, 2018
- HyLAW project, Hydrogen Law and removal of legal barriers to the deployment of fuel cells and hydrogen applications. Grant agreement No 737977. End Dec. 2018
- Global headline energy data from IEA (2016)
2 Details on the potential for hydrogen in Europe from the pre-study
2.1 The climate change challenge and European status
The average global surface temperature is used as a key indicator to measure the state of our climate. In 2018, the average temperature was 0.97 °C above the pre-industrial level[6]. From 2015 to 2017, the average temperature was more than 1.0 °C above normal.
The Paris agreement, reached in December 2015 and ratified in November 2016, constitutes a global effort to keep the global temperature increase in this century well below 2 °C above pre-industrial levels and to pursue efforts to limit this increase to 1.5 °C.
In 2018, IPCC launched its special report on the impacts of global warming of 1.5 °C above pre-industrial levels. The report presents scientifically based information on the global benefits of limiting the global average temperature to 1.5 °C, and states that “Global warming is likely to reach 1.5 °C between 2030 and 2052 if it continues to increase at the current rate.” Further, the report shows that the impact on sustainable development, poverty eradication and inequalities reduction would be greater if global warming were limited to 1.5 °C rather than 2 °C, provided that certain measures were taken during the decarbonization phase.
For the EU, leading the world towards climate neutrality means achieving it by 2050[7]. In 2016, total greenhouse gas emissions in Europe amounted 4300 Mt CO2 eq., a reduction of 24 % compared 1990 emissions. As illustrated in Figure 4, this corresponds to an average reduction of 50 Mt CO2 eq. per year over the period. With a goal set for a climate neutral economy in 2050, an average reduction of 130 Mt CO2 eq. per year from 2017 to 2050 is required.

The Baseline scenario of A Clean Planet for all, shown in Figure 4 by its emission prediction for 2050, represents the current decarbonization trajectory of EU. The scenario was included to model the GHG emission reductions resulting from agreed EU policies and policies that had been proposed by the Commission and were under discussion in the European Parliament and Council at the time of the development of the document. The scenario results show that significant additional measures are needed to reach a goal of climate neutrality in 2050.
As presented in Figure 5, the EU GHG emissions are mainly found in the sectors of public electricity and heat generation (29 %), road transport (25 %), manufacturing industries and construction, petroleum refining, cement and steel production (20 %) and residential, commercial and institutional (15 %)[8].

2.2 Emissions related to production of hydrogen from electricity and from natural gas with CCS
A comparison of the CO2 intensity of hydrogen production from EU average grid electricity and from natural gas with CCS is presented in
Figure 2. In 2016, the average emissions per MWh generated were 296 kg CO2 in the EU countries[9]. Production of hydrogen from electricity with such a CO2 intensity would result in an emission rate of 393 g CO2 per kWh (based on the higher heating value) of produced hydrogen[10]. If the hydrogen was produced from natural gas with average European up- and mid- stream CO2-emissions, combined with CCS, the emission rate would be 51 g CO2 per kWh (based on the higher heating value) of produced hydrogen. This assumes 80 % conversion efficiency and a cost-effective capture rate of CO2 at 94 %. Hence, the CO2-emissions are 7.7 times lower for hydrogen produced from natural gas with CCS than for hydrogen produced from the grid electricity.
Outlooks from “A Clean Planet for all”[11] and IRENA’s Renwable Energy Prospects for the European Union[12], give a corresponding ratio in the range of 5.3 to 5.6 for 2030, compared to 7.7 for 2016. Hence it is reasonable to assume that well beyond 2030 the hydrogen with the lowest carbon intensity would be produced from natural gas with CCS.
The estimate for 2050 is made from the most ambitious scenario presented in “A Clean Planet for all” which reaches climate neutrality in 2050. It shows that by this time, the carbon footprint of electricity production is small enough to produce hydrogen with least emissions. The precise timing for this transition is uncertain and will depend on the pace of deep decarbonization of the grid post 2030. It is uncertain when hydrogen production from natural gas with CCS and electricity are equally clean. On-going efforts to reduce the CO2 emissions up- and midstream for natural gas, as well as for the hydrogen production with integrated CCS, will prolong the period where natural gas-based hydrogen has the least emissions. These estimates are partly included, e.g improved carbon capture ratio of 96 % and a reduction in up-and midstream emissions of methane of 33 %[13] for the emissions in 2030 and 2050 in line with the first collective methane target for member companies of the Oil and Gas Climate Initiative. Furthermore, the presented emissions only include direct emissions of electricity generation. Indirect emissions in the electricity sector (e.g. construction) are not accounted for in this picture.
2.3 The potential for hydrogen in Europe – the pre-study estimate
The estimated potential for hydrogen in Europe, as presented in Section X have been made using statistical energy information from IEA, the baseline predictions for 2050 from “A Clean Planet for all” by the European Commission, and outlooks from Hydrogen Roadmap for Europe. The estimates have been made with as few assumptions as possible to allow for the readers to assess the values as transparently as possible since in practice, the degree of realization of the potential will most probably be somewhat lower and it will be different for each sector.
In brief, the following assumptions have been made for each of the sectors for 2050:
In the following paragraphs, a more detailed overview of the basis for the estimated potential for hydrogen in Europe. The estimates have been based upon the current energy demand per sector and prospects for each sector. The energy use in 2014, as reported by IEA is shown in Figure 6. The fuel consumption for electricity and heat are estimated based upon data from Eurostat for 2014, as shown in Table 1. In the following it is assumed that hydrogen can replace all fossil fuels in production of heat. The same assumption may be made for electricity production. However, as renewable energy sources as sun and wind to a large degree is converted to electricity first, it is expected that renewably based electricity production will replace large share of the fossil sources with natural gas serving as fast balancing energy source. Hydrogen can replace natural gas in the power section as clean energy source.
Table 1: Fuel consumption (in TWh) for electricity and heat in 2014 (Eurostat, 2018)
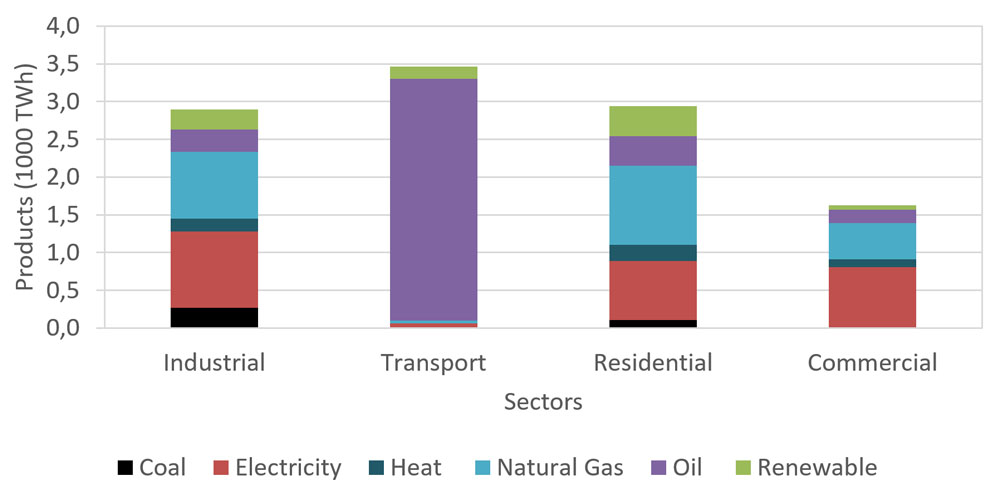
2.3.1 The potential for hydrogen in the industry sector
Figure 7 shows the historical development of energy consumption in industry. Although the energy consumption was reduced by 27 % from 1971 to 2014, this reduction is not equal for all energy carriers. Especially oil and coal usage were reduced by 82 % and 75 % respectively, whereas electric power consumption increased by 60 %, natural gas consumption by 105 %, and renewable energies by 422 %. Due to the variety of applications (heat, feedstock, reduction agent), it is not straight forward to predict the future development. In “A Clean Planet for all”, industrial energy consumption is reduced by 23 % in the baseline scenario due to improved feedstock utilization and energy efficiency.
The potential for clean hydrogen in the industry sector can be separated into three different fields (see also FCH JU Hydrogen Roadmap Europe and the introduction):
- Substitution of existing hydrogen applications with clean hydrogen;
- Delivery of medium- and high-grade heat to industrial process;
- As reduction agent in steel making.
Current application of hydrogen can be found mostly in the chemical industry. Here, hydrogen is used as feedstock for a large magnitude of reactions in refineries and in the ammonia production. Furthermore, the production of methanol is entirely based on natural gas-derived hydrogen. Currently, the majority of hydrogen is produced using natural gas reforming without CO2 capture. A potential hydrogen infrastructure allows the application of clean hydrogen. According to the FCH JU report “Hydrogen Roadmap Europe”, the current application of hydrogen in refineries and ammonia production in Europe corresponds to 282 TWh. Assuming an energy efficiency from methane to hydrogen of 80.0 % (due to the utilization of methane for providing heat to the endothermic reaction), this corresponds to 352.5 TWh of natural gas. Substituting this demand by clean hydrogen will correspond to annual CO2 reductions of 60 Mt CO2, a reduction of 80 %. We assume that the overall consumption of hydrogen will remain the same as today. This can be reasoned by increased ammonia production to feed the growing world population with reduced usage of hydrogen in refineries due to reduced need for fossil fuels.
Medium- and high-grade heat is currently mostly provided by burning oil, natural gas, or coal. Here, clean hydrogen can provide a route for the decarbonization. In a first step, addition of hydrogen to natural gas may result in a simple method for emissions reductions and development of hydrogen as heat source. In subsequent steps, hydrogen can be the only fuel source for providing medium- and high-grade heat. Estimates for heat usage are included in the defined cases. We assume that all provided heat (see Figure 6) now derived from fossil sources is substituted with hydrogen and that 40 % of current natural gas consumption is related to providing high- and medium-grade heat and will remain at this level in 2050. This corresponds to a hydrogen potential of 470 TWh and a CO2 reduction potential of 85 Mt CO2. Contrary to high- and medium-grade heat, low grade heat can be provided using heat pump. Applying hydrogen would result in a large exergy destruction.
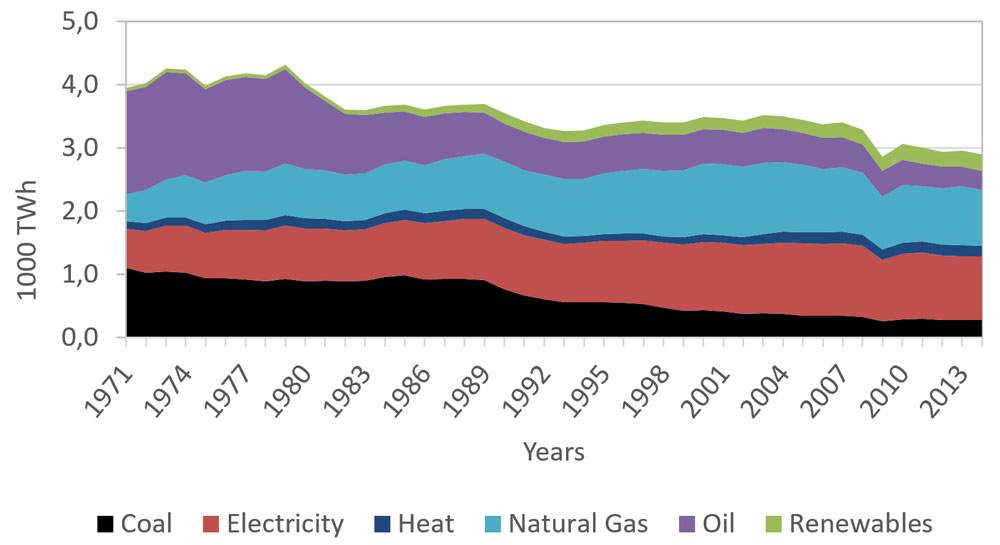
In addition to the provided heat and heat from natural gas burning, heat is as well generated from both coal, oil, and waste. The cement industry is one example of an industry requiring large amount of high-grade heat, around 5-7 % of industrial energy demand. The heat demand of the cement industry is estimated at 117 TWh in 2012, of which 40 % is provided by alternative fuels, e.g. biomass and waste, whereas the rest is provided mostly by coal (Ecofys, 2017). The utilization of alternative fuels is expected to increase to 60 % on a medium-term outlook. Hence, we assume that 40 % of the energy demand of cement production can be provided by hydrogen, that is hydrogen substitutes coal in the heating. According to the “Technology Roadmap: Low-Carbon Transition in the Cement Industry” of the IEA, the energy demand will remain roughly the same in 2050 as today. This is reasoned by an increased production volume and improved energy efficiency. Combined with 40 % of the energy provided by hydrogen, this corresponds to 44.8 TWh hydrogen and a CO2 reduction potential of 15 Mt CO2. Note, that 2/3 of the emissions associated to cement production are process emission and changing the energy source for heating does not influence these emissions.
Currently, only 1 % of steel is produced using hydrogen as reduction agent in direct reduction furnaces. Here hydrogen has a large potential for reduction of carbon emissions. FCH JU Hydrogen Roadmap Europe report reported an emission reduction by 66 % and a potential of 20 % of the steel manufacturing in 2050. This corresponds to a hydrogen potential of 140 TWh of hydrogen and a CO2 reduction potential 46 Mt CO2 when the emissions for hydrogen production are included.
2.3.2 The potential for hydrogen in the transport sector
In the transport sector, hydrogen has the largest potential in replacing fossil fuels in the heavy-duty segment, for applications like heavy-duty vehicles and in the maritime sector. Currently, data for the maritime sector has not been collected, and we will therefore focus on road-based transport. The maritime sector should however be included in future work.
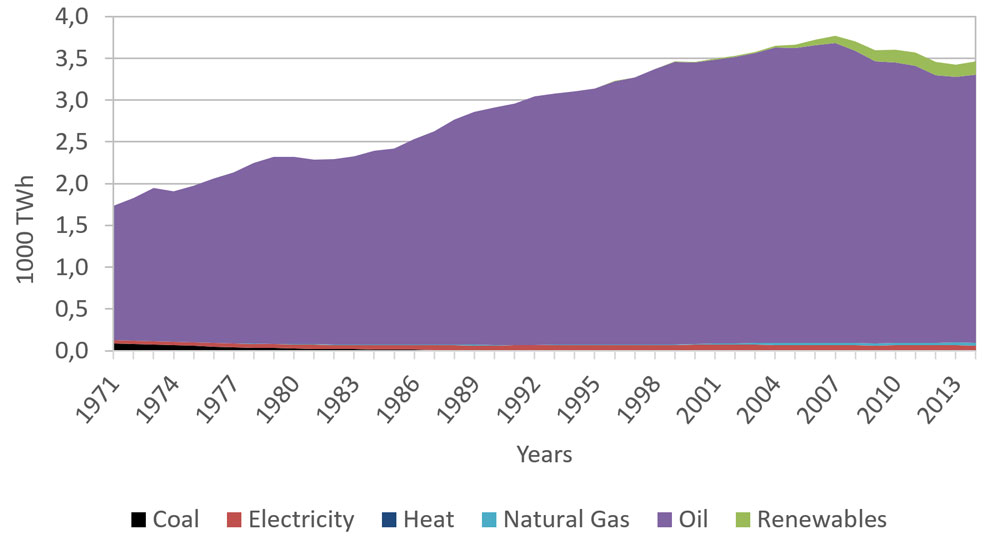
The road-based transport consumed 3,365 TWh in 2014 and based on the historical development shown in Figure 8 a complete utilization of oil is assumed. In “CO2 emission standards for heavy-duty vehicles”, the European Commission states that the share of emissions from heavy-duty vehicles amounted to 27 % of the road transport emissions in 2016, and that the share will increase without new policies. It is thus assumed that the share of energy consumption by heavy-duty vehicles is approximately equal to 27 %, and zero-growth in energy demand is used as a conservative approach. This implies a potential for hydrogen in 2050 equal to 409 TWh, and a corresponding potential for reduced emissions of 271 Mt CO2.
The rail sector corresponds in 2015 to 0.5 % of the emissions in the transport sector which is equivalent to 5.85 Mt CO2. Assuming that trains use diesel for propulsion and a similar efficiency for diesel engines as for fuel cells, this would correspond to an energy demand of 23 TWh hydrogen and a CO2 reduction potential of 4.69 Mt CO2. With higher efficiencies of hydrogen trains, the emission reduction increases, and the demand of hydrogen decreases. Currently, hydrogen trains have an efficiency of around 50 % compared to diesel-electric trains of around 50 %. Substitution of electric trains with hydrogen trains is unlikely as the infrastructure for electric trains is existing and hydrogen trains are less efficient due to the production of hydrogen and the conversion to electricity in a fuel cell. Both processes are less efficient than using the electricity directly.
2.3.3 The potential for hydrogen in the residential and commercial sectors
The historic development of energy usage in the residential and commercial sectors are shown in Figure 9 and Figure 10. The Baseline scenario of “A Clean Planet for all” is used as a basis for predicting the energy consumption in 2050. The scenario represents the development of the energy system of EU given the implementation of “agreed EU policies, or policies that have been proposed by the Commission but are still under discussion in the European Parliament and Council”. In this scenario, the residential and service sectors have a change in final energy consumption of -38 % and -15 %, respectively, from 2005 to 2050.
The use of fossil fuels in the two sectors is probably entirely for heating purposes. Hence, it is assumed that hydrogen can be used to replace the fossil fuels consumed, including the fossil fuels used to generate the heat consumed (see Figure 9 and Figure 10). For the residential sector it is assumed that the entire change in final energy consumption is due to reduced demand for fossil fuels for heating. For the commercial sector it is assumed that the need for electricity and heat (non-electricity in Figure 10) is reduced equally. The deviation in assumption for residential and commercial sectors is based upon the comparably larger share of electricity consumed in 2014 in the commercial sector. A reduced consumption of fossil fuels is thus estimated to -47 % and -16 % for the residential and commercial sectors, respectively. In total this amounts to a predicted potential for H2 of 1,503 TWh in 2050. If the entire potential is implemented, this equals a total reduction in GHG emissions of 301 Mt CO2.
Note, that the current application of coal and oil in the residential sector is in regions with limited access to a natural gas grid, and hence, a potential hydrogen grid in the future. However, based on the historic reduction in consumption of oil and coal in the residential sector, it can be expected that both energy sources will be substituted by either electricity or hydrogen in the future, both requiring investment into infrastructure.
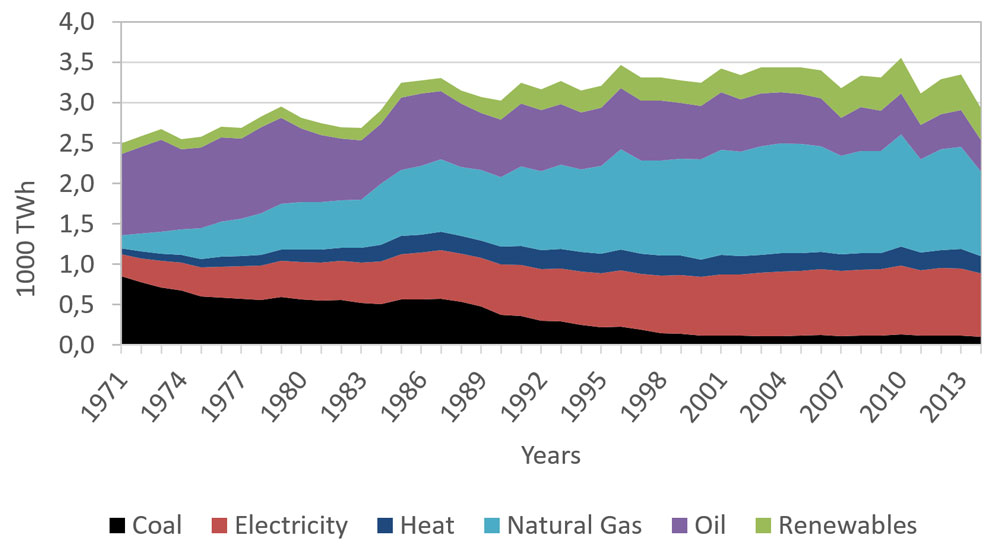
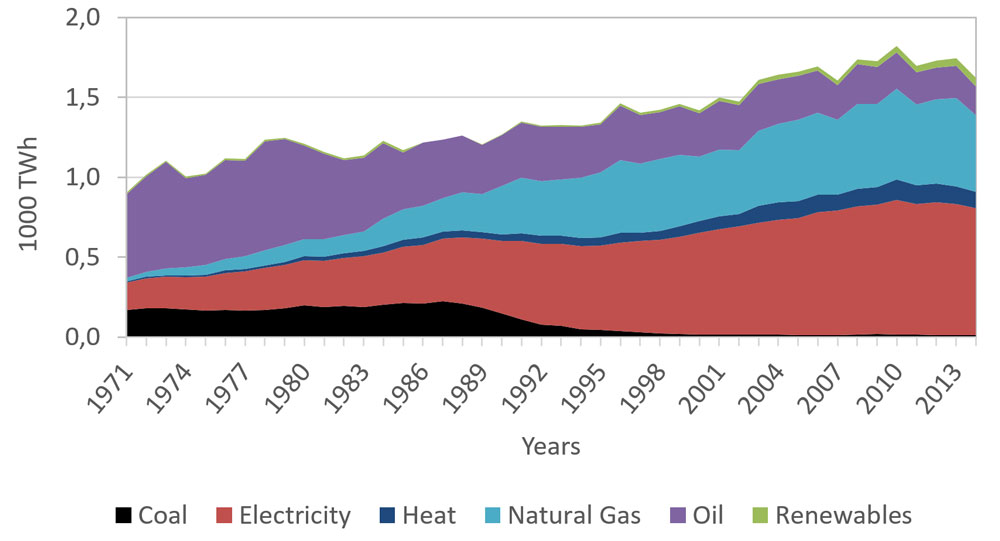
2.3.4 The potential for hydrogen in the power sector
Hydrogen can be used as decarbonised energy source in the power sector. For example, Equinor and Vattenfall are investigating the utilization of hydrogen at the Magnum combined cycle gas power plant in the Netherlands[14]. The advantage of using hydrogen compared to natural gas with CCS is a faster response to the changes in the power demand as a gas turbine allows faster ramping of power output without a coupled carbon capture unit. The total annual natural gas demand in the power sector varies in “A Clean Planet for all” from 70 TWh in the energy efficiency scenario via 360 TWh in the 1.5C Tech scenario to 930 TWh in the baseline scenario.
We assume that 50 % of the natural gas for power generation in the 1.5C Tech scenario is substituted with hydrogen. This corresponds to an annual potential of 180 TWh and a CO2 reduction potential of 29 Mt CO2 if the power production with natural gas does not include CCS. This is 1.8 times higher than the assumption in the FCHJU report. However, it is still lower than today’s consumption of natural gas for the power production as outlined in Table 1.
2.4 Hydrogen production from biomass as a GHG removal technology
Production of hydrogen from biomass (including biogas) with CCS is a greenhouse gas (GHG) removal technology. Using a mixture of already available biogas and natural gas as feedstock gives climate neutral or even climate positive hydrogen within the next years. Biomass is however a limited resource due to area limitations and the need for food production. Knowledge on the exact potential for biomass available for hydrogen production is scarce due to competing application areas like biofuels, heating, and power generation.
To illustrate and provide an example of the scale of possible hydrogen production, it is assumed that 20 % of the total amount of biomass in the Baseline scenario of “A Clean Planet for all” can be used. All other scenarios in “A Clean Planet for all” (except for 1.5LIFE-LB) assume significantly higher energy available from biomass (12-49 % more than Baseline). This indicates, that the potential for hydrogen from biomass could be higher.
In order to estimate the scale of the captured CO2 relative to the needed EU efforts to remove of CO2 from the atmosphere, a rough assessment has been made. The estimated production of hydrogen from biomass with CCS results in removal of CO2 from the atmosphere of 147 Mt CO2 per year in 2050[15]. This would cover approximately 57 % of the demand for CO2 removal in the 1.5 °C Technical scenario (1.5TECH) of “A Clean Planet for all”. This is the 1.5 °C scenario with significant BECCS. Additional removal could potentially be obtained through e.g. CCS combined with biomass currently used for power generation or direct air capture with storage of CO2. The estimate thus indicates that the volumes of CO2 that must be removed from the atmosphere annually by 2050 to reach a climate neutral economy in EU are feasible and hydrogen produced from biomass with CCS could play a key role.
2.5 A scenario for future production of hydrogen from natural gas, electricity from renewables and biomass
Figure 11 shows indicative hydrogen production from natural gas, natural gas with CCS, biomass and CCS, and electrolysis using renewable power – detailed pathways should be developed in a future, more comprehensive study. The scenario indicates how hydrogen from the different sources can support the development of a large-scale market for hydrogen, rather than being competitors.
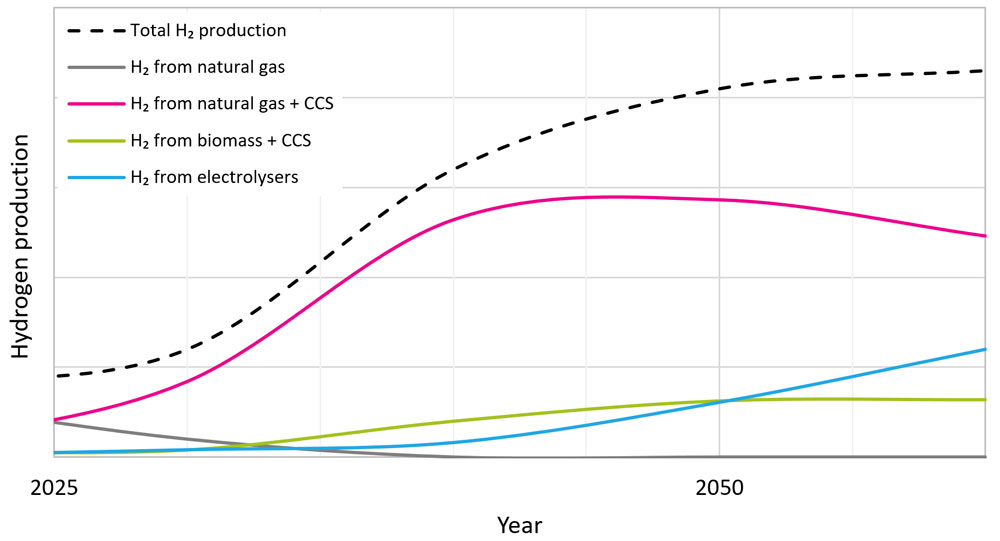
According to the Hydrogen Roadmap Europe, 325 TWh of hydrogen was produced in Europe in 2015. Fossil fuels as feedstock dominate the world market as 96 % of hydrogen is produced by fossil sources (IEA, 2015). Natural gas accounts for 48 % of the total production volume, electrolysis to 4 %. It is thus assumed that all hydrogen initially is produced from unabated natural gas (grey curve). The volumes of hydrogen produced from natural gas with CCS (pink curve) must be seen in relation to the current consumption of natural gas and the potential for hydrogen as outlined in Section 0 and section 2.3. In 2016, Europe consumed 4435 TWh natural gas. To give an indication of potential scale, if all this natural gas was replaced with hydrogen produced from the natural gas with CCS, a total amount of 3562 TWh of hydrogen could be produced and the European emissions could be reduced by 875 Mt CO2 per year. The potential for hydrogen presented in Section 0 implies an annual consumption of 3050 TWh of hydrogen. In Figure 11, it is assumed that hydrogen from natural gas with CCS covers approximately half of the potential for hydrogen in 2050.
The availability of the biomass, as presented in Figure 11, is based upon the Baseline scenario of “A Clean Planet for all”. As described in Section 2.4, biomass is a limited resource and there is a scarce knowledge on the exact potential for biomass available for hydrogen production. The estimate presented in Figure 11 is made according to our best knowledge, and to illustrate and provide an example of the scale of production assuming that 20 % of the total amount of biomass in the Baseline scenario can be used for hydrogen production. All other scenarios in “A Clean Planet for all” (except for 1.5LIFE-LB) assume significantly higher energy available from biomass (12-49 % more than the baseline). This indicates, that the potential for hydrogen from biomass can be higher.
Production of hydrogen from renewably based electricity must be based upon the estimated future curtailment of renewable electricity, which will be a major driver for the business model. The exact amount of urtailment is depending on power grid investments, priority dispatch, and increase in renewable power generation. Currently, the knowledge on the future development of the curtailment is scarce. In its 2030 outlook for renewable energy, IRENA predicts a curtailment of electricity generation from renewable energy sources of 2 %. However, 2018 curtailment rates in Europe are as example 4.3 % in Germany (5.4 TWh) and 6 % in Ireland and Northern Ireland (707 GWh).
It is assumed that production of hydrogen will not be economically feasible for curtailment rates at these levels due to the low utilisation time of electrolysers. The estimate presented in Figure 11 is based upon the assumption that there is a business case for hydrogen from renewably based electricity from 2040 onwards, with only smaller volumes of hydrogen being produced before this. Further it is assumed that 10 % of the electricity consumption of the Baseline scenario of “A Clean Planet for all” is used for hydrogen production in 2050. Both these assumptions are made according to our best knowledge and should be assessed more in detail in further work.
The presented scenario for hydrogen production shows that there is a potential for production of large volumes of hydrogen based upon renewable sources in the long-term but that hydrogen from natural gas with CCS is dominant in the nearer term. Currently, gasification of unconventional biomass is still on pilot scale. Further, despite increasing renewable power generation, hydrogen production from renewable power sources through electrolysis is only commercially viable in niche applications and shows limited production capacity. Hence, until the cost of these technologies is reduced, clean hydrogen can be produced from natural gas with CCS in sufficient quantities to supply a European hydrogen market and gradually replace existing production of hydrogen from natural gas without CCS. This shows that the technologies for hydrogen production are complementary instead of competing.
2.6 Barriers to the use of hydrogen from natural gas
Numerous existing European legislative acts are relevant to the deployment of hydrogen as a product and in fuel cell technologies. Some legislative acts impact hydrogen technology deployment indirectly, such as health and safety, environmental, labour and transport laws. EU legislative acts are often a source of obligations for developers and manufacturers, and the extent to which they represent a barrier to hydrogen deployment depends mainly on national implementation which differs across countries and on involuntary mismatches between rules imposed at the national level (e.g. standards for fuel quality and measurement).
Hydrogen in the gas grid
Existing legal and administrative barriers to the injection of hydrogen into the gas grid are of high severity. These barriers are of a structural type, preventing the injection of hydrogen in the gas grid and Power-to-Gas facilities.
The regulatory framework has been drawn up around natural gas, and specifically, the quality standards are based on gas calorific value, or the Wobbe Index. Adding hydrogen to the gas stream impacts calorific value, flow properties, density and flame speed as well as pipeline materials and gas grid operations.
Widely varying national limits for hydrogen concentrations in the gas grid exist in Europe, and hydrogen injection permitting is considered on a case-by-case basis (Table 2).
Table 2: Legal framework for H2 levels in the gas grid (HyLAW project).
European coordination appears necessary to validate gas grid operation where hydrogen thresholds are significantly higher (such as in DE, FR, NL & UK). Gas Appliance Directive and Gas Appliance Regulation revision will be necessary to allow higher hydrogen concentrations in the gas grids.
Barriers in residential and commercial buildings
An EU appliance assessment at the end-user level is essential to the large-scale deployment of hydrogen.. For example, there is an identified need to define the acceptable safety and operational threshold of end-user appliances at the domestic, commercial and industrial levels.
When new investments at the end-user side are needed to use hydrogen, e.g. in appliances able to use hydrogen, a supply chain assessment of global economic impact to identify additional costs at different actors’ level and targeted incentives for the deployment of hydrogen will be necessary.
High economic barriers exist for stationary power in residential and commercial buildings (micro-Combined Heat and Power – CHP) due to a lack of financial incentives.
The legal framework for permitting Power-to-Gas plant and grid connection/injection requirements between the hydrogen supplier and the gas grid operators should be included within EU regulatory frameworks to ensure comparable treatment across the EU.
Gas Appliance Regulation revision will be necessary to hydrogen tolerant gas appliances.
Barriers in the transport sector
Road vehicles face barriers mainly associated with a lack of incentive policies and infrastructure investments.
The barriers in the production, stationary storage and use of hydrogen as a fuel in Hydro Refuelling Stations (HRSs) are not negligible, because the permitting process is long, costly and uncertain due to a lack of clear rules and procedures. Only Germany, Denmark, the UK and the Netherlands currently have rules to regulate the permitting of HRSs.
A potential conflict has been identified with the gas composition of the gas grid required for fuel supply to CNG vehicles. For CNG vehicles, the hydrogen limit is maximum 2% according to UNECE regulation R1106. This means that if a CNG fuelling station is connected to the gas grid, the admissible hydrogen concentration for that local grid must not exceed 2 vol%.
Other barriers in relation with HRSs are the overlap of various responsible authorities, a lack of administrative practice, and a lack of guidance provided to operators. The permitting requirements applicable to HRSs draw on obligations established at EU level, such as risk assessments (SEVESO Directive), health and safety requirements and conformity assessment procedures, (ATEX Directive), integrated environmental obligations (Industrial Emissions Directive – IED), and environmental impact assessment procedures (Strategic Environmental Assessment – SEA and Environmental Impact Assessment – EIA Directives).
Finally, hydrogen as a fuel for road vehicles lack consistent incentive policies, affecting its large-scale deployment.
In the case of hydrogen vessels (maritime and inland waterways), major regulatory barriers were identified. Maritime and inland-waterway transport face very high legal and administrative barriers to hydrogen, such as IMO regulation aiming to reduce CO2 emissions by 50% by 2050 and imposing a 0.5% sulphur cap on marine fuel from 2020. This could represent an opportunity for new alternative fuels including hydrogen power vessels, however, “type approval” of hydrogen fuel cell vessels remains complicated due to the absence of rules.
Hydrogen specific requirements are not yet on the agenda in the International Maritime Organization/ Carriage of Cargoes and Container (IMO/CCC). For inland vessels, Directive 2016/1629/EU empowers CESNI (Comité Européen pour l’Élaboration de Standards dans le Domaine de Navigation Intérieure) to develop standards in the field of inland navigation. It is crucial for all actors to act in a coordinated manner at the IMO level to develop specific regulations for hydrogen.
In conclusion, many of the barriers to hydrogen deployment are a result of regulatory gaps caused by a lack of harmonization of rules and approaches at the European level. A growing body of EU law references hydrogen directly, and have a major impact on the deployment of hydrogen technology, especially on the use of hydrogen as a fuel.
Across the EU, barriers are present in all countries but they exhibit varying degrees of severity. In all cases, actions are necessary to unlock the full potential of hydrogen technologies in all countries and at the EU level. Relevant authorities should review technical and gas composition rules to establish legal pathways to support Power-to-Gas operations and increased hydrogen use in transmission and distribution gas networks.
A coordinated EU-wide review of safety and technical integrity limitations for hydrogen connection and injection into the gas grid is essential.
2.7 References
- A Clean Planet for all by the European Commission (2018).
- Annual European Union greenhouse gas inventory 1990–2016 and inventory report 2018 from EEA (2018)
- Energy statistical datasheets for the EU countries from Eurostat (2018)
- Hydrogen Roadmap Europe by FCH-JU (2019)
- HyLAW project, Hydrogen Law and removal of legal barriers to the deployment of fuel cells and hydrogen applications. Grant agreement No 737977. End Dec. 2018
- Global headline energy data from IEA (2016)
- Technology Roadmap: Hydrogen and Fuel Cells by IEA(2015)
- H21 North of England (2018)
- Renewable Energy Prospects for the European Union, IRENA, 2018
[1] Source: Energy statistical datasheets for the EU countries from Eurostat [2] EEA CO2 emission intensity; accessed on 31.05.2019 [3] Assuming production of hydrogen with a final state of 20 bar. [4] All scenarios have a similar grid intensity of approximately 168 kg CO2/MWh grid intensity in 2030. [5] REmap case, which assumes a grid intensity of 177 kg CO2/MWh in 2030. [6] Global Climate Report - Annual 2018, https://www.ncdc.noaa.gov/sotc/global/201813. Pre-industrial level is the average global surface temperature of the years 1880 to 1900. [7] Press release, "The Commission calls for a climate neutral Europe by 2050", November 2018. https://europa.eu/rapid/press-release_IP-18-6543_en.htm [8] Data for 2016. Source: Annual European Union greenhouse gas inventory 1990–2016 and inventory report 2018 from EEA. [9] CO2 emission intensity, electricity generation, EEA; accessed on 31.05.2019 [10] Assuming production of hydrogen with a final state of 20 bar. [11] All scenarios have a similar grid intensity of approximately 168 kg CO2/MWh grid intensity in 2030. [12] REmap case, which assumes a grid intensity of 177 kg CO2/MWh in 2030 [13] Oil and Gas Climate Initiative sets first collective methane target for member companies https://oilandgasclimateinitiative.com/oil-and-gas-climate-initiative-sets-first-collective-methane-target-for-member-companies/ [14] https://www.equinor.com/en/news/evaluating-conversion-natural-gas-hydrogen.html.html [15] Assuming 80 % energy efficiency from biomass to hydrogen, a CO2 intensity of biomass of 0.4 kg CO2/kWh, and a carbon capture ratio of 93 %.
Comments
That’s a really insightful pre-study, Gunhild! Agree with your points about hydrogen being so much cleaner than other sources of energy. In fact, it is estimated that a shift to zero-carbon hydrogen in existing applications could reduce global energy-related CO2 emissions by 7%.
That’s a great summary Gunhild! There are certain challenges regarding scale and source when it comes to hydrogen. But the benefits far outweigh them. They are an extremely clean source of energy. Around one-fifth of all greenhouse emissions in America are a result of fossil fuel powered vehicles. With hydrogen, this can be drastically reduced.
Thank you. Indeed, the benefits of hydrogen pushes towards overcoming the obstacles. As far as we can see, hydrogen holds the potential to reduce emissions in the transport sector as well as for industry, heating of the building stock and the power sector.